The Creation of the Universe’s Heaviest Elements Through Star Collisions
Scientists have new evidence about how cosmic cataclysms forge gold, platinum and other heavy members of the periodic table
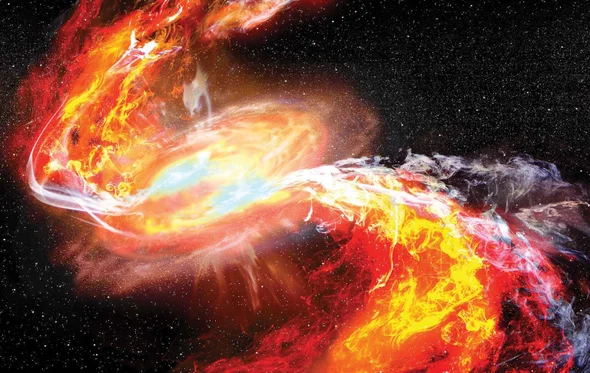
Star fragments can be found both outside of us and inside of us. Some of the most intense explosions in the universe are where around half of the abundance of elements heavier than iron comes from. These substances ultimately find their way to Earth and other planets as the cosmos expands and new stars and planets are created from old gas and dust. Humans and several other animals have become dependent on them in our bodies and in our daily lives after 3.7 billion years of evolution on our planet.For instance, iodine is a part of the hormones required to regulate our metabolism and control brain growth. Strontium is used by a kind of marine microplankton known as Acantharea to build complex mineral skeletons. Our laptop displays and the processors in our cellphones depend on gallium. Additionally, the JWST’s mirrors are plated with gold, a material chosen for its inertness and capacity to reflect infrared radiation (not to mention its popularity in jewelry).
Although scientists have long had a general understanding of how these atoms form, the specifics have been the subject of intense disagreement. That changed recently when astronomers saw heavy-element synthesis in operation for the first time. The data implies that the procedure went somewhat like this.
A neutron star, one of the weirdest things in the cosmos, was created long ago when a star more than ten times as big as the sun perished in a cataclysmic explosion. A relic of the central core that had been squeezed to extremely high densities, where matter may take on shapes that we do not comprehend, was this brand-new star. The neutron star’s journey may have come to an end if it had continued to cool in the depths of space.However, the majority of large stars have a twin and exist in binary systems; as a result, their partners eventually experienced the same fate as our initial star, leaving two neutron stars in a mutual orbit. The stars spiraled in in a dance that lasted for ages, at first slowly and then quickly. Tidal forces started to tear them apart as they got closer to one another, sending neutron-rich stuff into space at speeds near to one-third the speed of light. Finally, the stars came together, blasting shockwaves through the cosmos and igniting cosmic fireworks throughout the whole electromagnetic spectrum.
The dinosaurs lived on our own pale blue planet at the time of the collision, 130 million light-years distant in a peaceful region of the Milky Way. Gravitational waves, which are ripples in spacetime, started spreading over the universe, and in the time it took them to travel the great distance to Earth, life on the planet changed significantly.
As civilizations developed and collapsed, new species emerged and went extinct, and people started to stare up at the sky out of curiosity, they created tools that could do amazing things like measure minuscule distortions in spacetime. The light from the merger and gravitational waves, which are moving at the speed of light, eventually collided with Earth. The presence of new elements was indicated by a peculiar light, which astronomers were able to identify. Heavy element production had recently been experienced by humanity.
As a specialist in cosmic catastrophes, I’m fascinated by this tale of the birth of something new and lasting, even valuable, from an ancient residue of a once-brilliant star. And I’m ecstatic that we can now witness it taking place. The discovery has generated a number of brand-new issues in astrophysics while simultaneously providing answers to certain long-standing ones. But I feel inspired, as do many other scientists. In a way that was previously unimaginable, our newly discovered capacity to detect gravitational waves as well as light from the same cosmic source promises to advance our understanding of astrophysical explosions and the synthesis of elements.
WE ARE STARDUST
The search for an explanation of the genesis of heavy elements is a component of a broader scientific attempt to respond to the fundamental question, “Where did everything come from?” From a few minutes after the big bang until the present, the periodic table’s elements have had a cosmic history. Three minutes after the universe’s creation, the first three elements—hydrogen, helium, and lithium—were created. The earliest stars were created from these components, glowing brightly and fusing new elements in their cores both during their lifetimes and at their violent deaths.
The remnants of these explosions gave birth to the following generation of stars, which were enriched with the elements created by the initial stars. All the elements, from helium on the light end with two protons per atom up to iron with 26 protons in its atomic nucleus, are explained by this process, which is still going on today. The heaviest elements, like Tennesseeine, which has 117 protons, are entirely artificial. In particle accelerators, however, where they normally only exist for a few thousandths of a second before fading, scientists may push them into being.
A few decades ago, scientists proposed the r-process, also known as rapid neutron capture, as the mechanism responsible for the production of roughly half of the elements heavier than iron. The s-process, or slow neutron capture, which takes place in low-mass, long-lived stars, is assumed to be the source of the remaining particles.
One or more neutrons are added to an atomic nucleus during the r-process and the s-process, respectively. However, since the amount of protons in an element’s nucleus determines its identity, adding neutrons does not result in the creation of a new element. The result is a heavier isotope of the same element, with a nucleus that has a different number of neutrons but the same number of protons. This heavy isotope frequently undergoes radioactive decay.
A neutron will change into a proton by a process known as beta-minus decay, ejecting an electron as well as a neutrino, another kind of subatomic particle. A new element is created as a result of an increase in the number of protons in the nucleus of an atom.
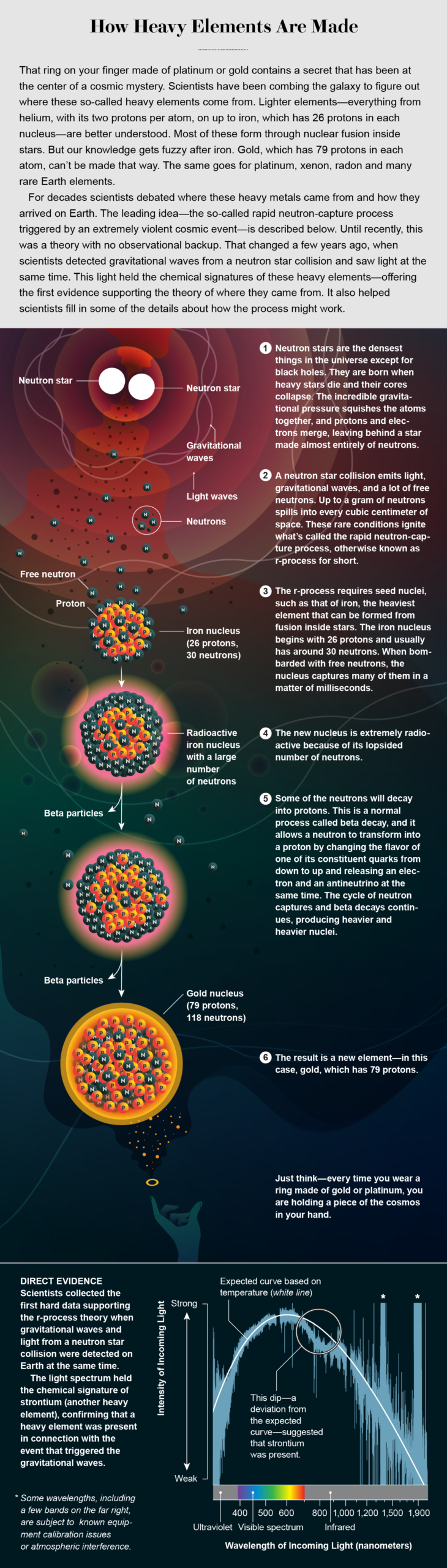
Speed is the primary distinction between the s-process and the r-process. In the s-process, neutrons are collected gradually by atoms, giving them plenty of time to decay into a proton and produce the next stable element in the periodic table—with only one more proton—before another neutron is present to be grabbed. Because the stars that support the s-process only have a tiny amount of additional neutrons hanging about, atoms are only infrequently able to grab new neutrons, therefore this process takes place across thousands of years.
The r-process, in comparison, has the ability to create the whole family of heavy components in a single stunning production that lasts just a fraction of a second. Neutrons are abundant in this situation and bombard nuclei one after another before they have a chance to disintegrate. When a nucleus reaches the so-called neutron drip line, which is the maximum neutron-to-proton ratio permitted by nature inside a nucleus, it can quickly inflate into an extremely unstable isotope.The highly heavy nucleus will eventually split into smaller nuclei or undergo beta decays, turning many of its neutrons into protons and creating a variety of stable heavy elements. There are several ambiguities over how this will go. Exotic nuclei that are unknown to science can form, for example, after a nucleus receives additional neutrons but before it becomes stable. It is challenging and occasionally impossible to measure the characteristics of these in-between nuclei in a laboratory setting because they push the boundaries of physics.
The r-process has been the subject of several theories throughout the years, but the reality has remained a mystery for more than 60 years, ranking among the biggest in nuclear astrophysics. They believed the r-process may be hosted by core-collapse supernovae, the cataclysmic deaths of stars with masses greater than eight to ten times that of the sun. The neutron richness and thermodynamic circumstances required, however, could not be replicated by simulations of ordinary core-collapse supernovae, with the possible exception of rare explosions triggered by powerful magnetic fields. It was proposed by James M. Lattimer and David N. Schramm in 1974 that the components for the r-process may be obtained from decompressing neutron star materials.
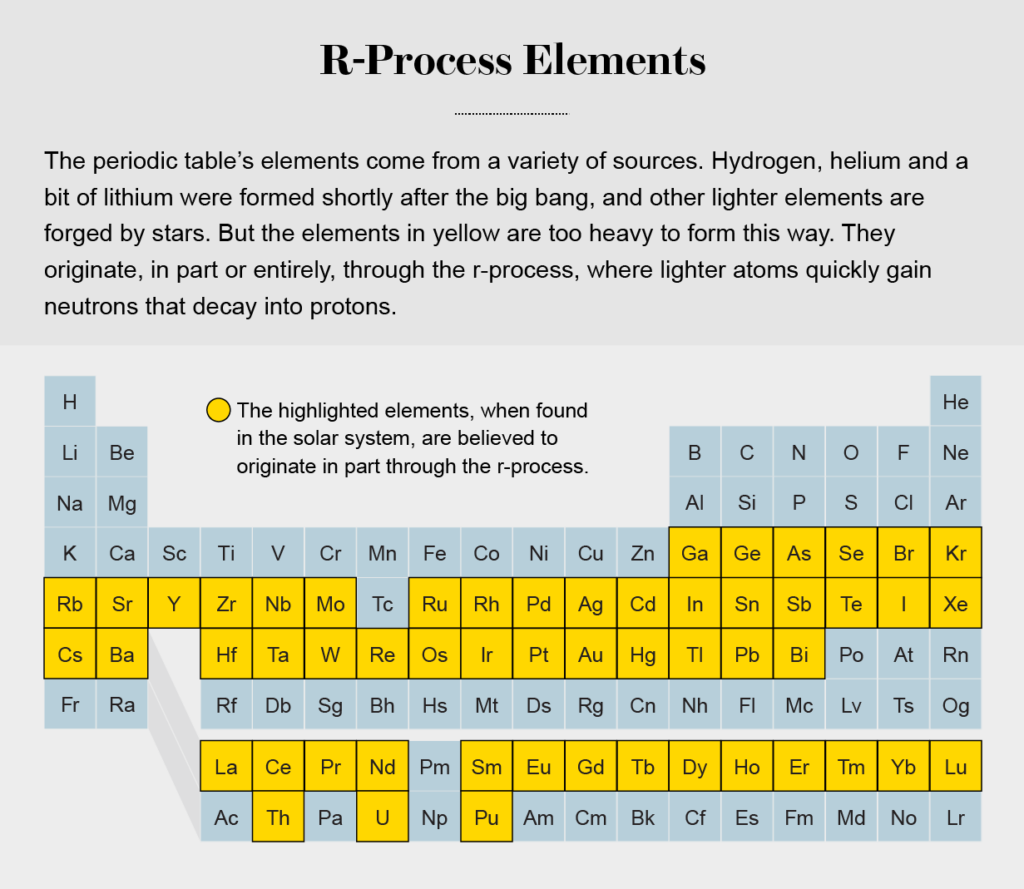
When a big star runs out of nuclear fuel and its gravity forces the core to collapse inward, a neutron star is created. The core of the star is compressed to extraordinarily high densities by the overpowering weight of the star, which causes protons and electrons to combine to form neutrons. The neutron star, a compact remnant containing the universe’s densest mass, survives the explosion while the remainder of the star is evacuated. More massive neutron stars continue to collapse into black holes, although the precise moment at which this happens and how “squishy” they are are unknown.
Neutron star core structures remain a mystery. They could have a crust of heavier nuclei at their surfaces that is largely made up of neutrons with a tiny amount of protons within. However, they may have even stranger interiors. From a soup of quarks and gluons, the particles that make up ordinary matter, to a sea of “hyperons,” which are formed of so-called weird quarks, matter may take on incredibly unusual shapes deep inside the neutron star.
The idea put out by Lattimer and Schramm was that when a neutron star collides with a black hole, neutron-rich matter is expelled. But by 1982, physicists were in favor of a scenario in which two neutron stars collide. Some scientists were trying to figure out how these collisions may create new elements, while others were attempting to forecast the type of light that would result from a neutron star merger. Gamma-ray bursts are very intense explosions in space that produce a flash of gamma rays. Some individuals hypothesized a link between neutron star collisions and gamma-ray bursts.
Additionally, since r-process nuclei would be unstable and subject to radioactive decay, they ought to be able to heat the material around them and generate an electromagnetic flare that would contain traces of the elements generated. After estimating that they would be around 1,000 times brighter than a typical flash of light known as a nova, Brian Metzger and his colleagues originally coined the word “kilonova” to describe such flares in 1998.
However, until a few years ago, when a unique combination of discoveries allowed researchers to peer right into the center of a neutron star merger, there had been little direct proof of this intensive theoretical development.
A COSMIC SYMPHONY
The Laser Interferometer Gravitational-wave Observatory (LIGO) made an astounding discovery in 2015 when it became the first organization to see gravitational waves, which are produced when two black holes spiral toward and merge. The detection bore the identification GW150914. I was a graduate student at NC State University at the time. I recall being moved to tears as I watched the announcement with the rest of the physics department in the common room of our building. I made an effort to learn everything I could about this brand-new portal to the cosmos. I discovered that neutron star mergers are harder to detect because they release less energy than black hole mergers. But I, along with other researchers, held out hope that they would eventually be discovered by the experiment.
After a few years, LIGO and its sister observatory Virgo discovered further binary black hole collisions. Neutron star mergers, however, were still elusive. Then, in the autumn of 2017, I learned that LIGO-Virgo had allegedly seen the first-ever neutron star collision. Rumors said that scientists had also seen a brief gamma-ray burst and an object that resembled a kilonova in addition to the gravitational-wave signal. There was a lot of enthusiasm among physicists.
I quickly became a witness to the announcement of the gravitational-wave detection, known as GW170817, and the related electromagnetic signals by scientists from LIGO and numerous sites across the globe. I was astounded by how much fresh information these observations had already produced. On arXiv.org, a website where academics may post early, unreviewed copies of their articles, there were over 70 additional publications regarding GW170817 posted the very following day. The occurrence foretold the potential of multimessenger astronomy, or the capacity to view cosmic occurrences via various “messengers” and integrate the data to gain a more comprehensive knowledge of the occurrence. For the first time, astronomers were able to see gravitational waves and light from the same astronomical source, including radio, optical, x, and gamma-ray rays.
Approximately 130 million light-years from Earth, a pair of neutron stars collided, producing the gravitational waves that LIGO-Virgo saw. Although it might look remote, this gravitational wave source is actually near by. The signal’s specifics, such as how the waves’ frequency and intensity fluctuated over time, allowed scientists to calculate that each neutron star had a radius of around 11 to 12 kilometers and weighed between 1.17 and 1.6 times the mass of the sun.
As soon as the gravitational-wave signal arrived, astronomers followed up with conventional telescopes. Working together, LIGO and Virgo narrowed the location range for GW170817 to a much smaller region of the sky than in previous gravitational-wave events. Roughly 1.7 seconds after the gravitational waves came in, gamma-ray telescopes Fermi-GBM and INTEGRAL detected a faint burst of gamma rays lasting only a couple of seconds that came from the same direction as GW170817.
For the first time, this research made a firm connection between neutron star mergers and brief gamma-ray bursts. There was nevertheless more! A new source of light was discovered in the ancient yet brilliant galaxy NGC 4993, as shown in images collected with the Henrietta Swopes one-meter telescope at the Las Campanas Observatory in Chile. Astronomers came to the conclusion that the signal was compatible with the hypothesis that heavy elements were being created there after dissecting the light into its component colors and analyzing its spectrum. A real kilonova was what we were observing.
It was fascinating to see how the spectrum of the kilonova evolved over time. Light with shorter, bluer wavelengths reached its peak first, while longer, redder wavelengths took over afterwards. The kind and speed of the material expelled from the merger can be used to explain these peaks. Fast-moving ejecta mostly composed of lighter heavy elements without any “lanthanides”—the metallic periodic elements from lanthanum to lutetium, which are particularly opaque to blue light—can create a blue kilonova. In contrast, slow-moving ejecta rich in heavy elements like lanthanides are needed for a red kilonova.
How does the merging produce these unique elements? We are now in theoretical and simulational area as a result of this inquiry. Researchers are currently working to comprehend the collision’s material ejection process, the composition of the material, and how the subsequent kilonova develops. Kilonova spectra are exceedingly challenging to separate. Because of how quickly the substance is flowing, different components’ fingerprints muddle and blend together. For many of the heavier elements, we also lack accurate atomic data, making it difficult to forecast what their spectral fingerprints would look like. Strontium has so far been the sole element that can reasonably be detected in the GW170817 kilonova spectrum. However, this is sufficient to demonstrate that the r-process occurred.
Years of theoretical predictions have been validated by the finding of this unique occurrence. Finally, astronomers have discovered a link between neutron star mergers and brief gamma-ray bursts. Heavy element signals may be seen in the kilonova spectrum, proving that neutron star mergers are at least one source of r-process elements.
But there is still a great deal to learn and learn about. Short gamma-ray bursts in mergers are produced by an unknown process. Neutrinos also affect the properties of stuff that is expelled during a merger in significant ways. Theoretical models must carefully follow these particles and their interactions, which is difficult and sometimes impossible due to computer power constraints. Additionally, we don’t know what resulted from the merger of the neutron stars. It may have been a black hole, a neutron star on its way to becoming one, or another neutron star. Finally, even while we now understand that the r-process may occur in neutron star mergers, this does not mean that this is the sole scenario.
Other scenarios, such as infrequent supernovae and neutron star collisions with black holes, are suggested by observations of extremely ancient stars with r-process elements. No matter how unusual the observation, we won’t be able to determine the source of heavy components. GW170817 is only the start.
NEW OPPORTUNITIES
It is unrealistic to anticipate that all kilonovae will resemble the one connected to GW170817. We anticipate seeing them in a variety of shapes and sizes, each with unique characteristics, and receiving numerous surprises. In fact, Northwestern University astronomers recently made the intriguing discovery of a kilonova with a lengthy gamma-ray burst, indicating that mergers may also produce gamma-ray bursts with longer light curves.
Experts from various fields will need to collaborate in order to understand the r-process, including observational astronomers who study both old and new stars, gravitational-wave astronomers who measure distortions in spacetime, nuclear theorists who build models of nuclear structures and of the matter inside neutron stars, experimental nuclear physicists who look for the characteristics of unstable neutron-rich nuclei, and computational astrophysicists who simulate events like neutron star mergers.
New telescopes will go online to gather light from the transitory sky as gravitational-wave observatories already in operation get more sensitive. New initiatives will analyze the nuclear characteristics of rare nuclei, such as the Facility for Rare Isotope Beams at Michigan State University, which will debut in May 2022. The ground-based Einstein Telescope is one of the proposed gravitational-wave observatories that are currently being built in Europe.
We are now able to examine the genesis of heavy elements in ways that were previously unattainable because to decades of advancement in several domains. Finally, the puzzle is about to come together. The periodic table contains all the isotopes of all the elements, and each one has the potential to reveal something about the universe’s nuclear past.
“This article was originally published with the title “Cosmic Alchemy” in Scientific American 328, 1, 30-37 (January 2023)
doi:10.1038/scientificamerican0123-30″
Do not forget to share your opinion with us to provide you with the best posts !
0 Comments