Astronomers Uncover Compelling Proof of a Pre-Big Bang Universe
The hot Big Bang is frequently presented as the Universe’s inception. However, there is a compelling piece of evidence that contradicts this notion.
The concept of the Big Bang dates back almost a century, originating when the initial evidence of the expanding Universe emerged. As the Universe continues to expand and cool today, it implies a past characterized by smaller size, higher density, and greater heat. In our contemplations, we can extrapolate backward to exceedingly minute scales, extreme densities, and scorching temperatures, ultimately arriving at a singularity where all of the Universe’s matter and energy were condensed into a single point. For many decades, these two aspects of the Big Bang—representing the hot, dense early Universe and the initial singularity—were intertwined and inseparable.
However, the 1970s marked the onset of scientists identifying certain enigmas associated with the Big Bang. They recognized several features of the Universe that could not be simultaneously explained within the framework of these two concepts. It was in the early 1980s when the theory of cosmic inflation was first proposed and developed, serving to dissociate the two interpretations of the Big Bang. It suggested that the early hot, dense state did not culminate in singular conditions but was preceded by a new inflationary state. Consequently, there truly existed a Universe prior to the hot Big Bang, and strong evidence from the 21st century substantially supports this assertion.
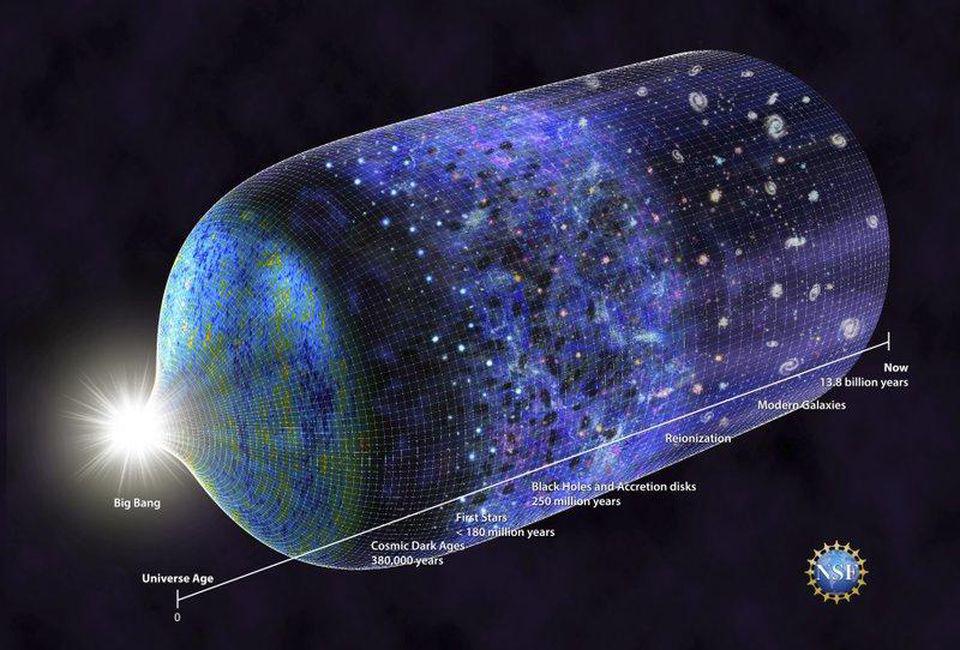
While we are confident in our ability to characterize the early Universe as hot, dense, rapidly expanding, and filled with matter and radiation—a concept known as the hot Big Bang—the question of whether this truly represents the Universe’s beginning can be addressed through evidence. The distinctions between a Universe that originated with a hot Big Bang and one that underwent an inflationary phase preceding and setting the stage for the hot Big Bang are subtle yet profoundly significant. To uncover the true origins of the Universe, we must seek evidence within the Universe itself.
The idea of the Big Bang dates back almost a century, arising when the initial evidence of an expanding Universe emerged. As we observe the Universe’s ongoing expansion and cooling, it implies a past characterized by smaller size, greater density, and higher temperature. Our imagination allows us to extrapolate backward to infinitesimally small scales, extreme densities, and scorching temperatures, ultimately reaching a singularity where all the Universe’s matter and energy converged into a single point. For many decades, these two concepts of the Big Bang, representing the early hot, dense state and the initial singularity, were closely intertwined.
However, in the 1970s, scientists began to identify certain puzzles related to the Big Bang, noting properties of the Universe that couldn’t be explained simultaneously within the framework of these two concepts. The introduction and development of the theory of cosmic inflation in the early 1980s separated these two interpretations of the Big Bang, suggesting that the early hot, dense state did not reach singular conditions but was preceded by a new inflationary phase. Thus, there truly existed a Universe before the hot Big Bang, and compelling evidence from the 21st century corroborates this assertion.
In a hot Big Bang scenario that we extrapolate back to a singularity, the Universe experiences extremely high temperatures and energies. Although the Universe has an “average” density and temperature, it also contains imperfections, with both overdense and underdense regions. As the Universe expands and cools, it also gravitates, leading overdense regions to attract more matter and energy over time, while underdense regions relinquish their matter and energy into the denser surroundings, laying the foundation for the eventual structure of the cosmic web.
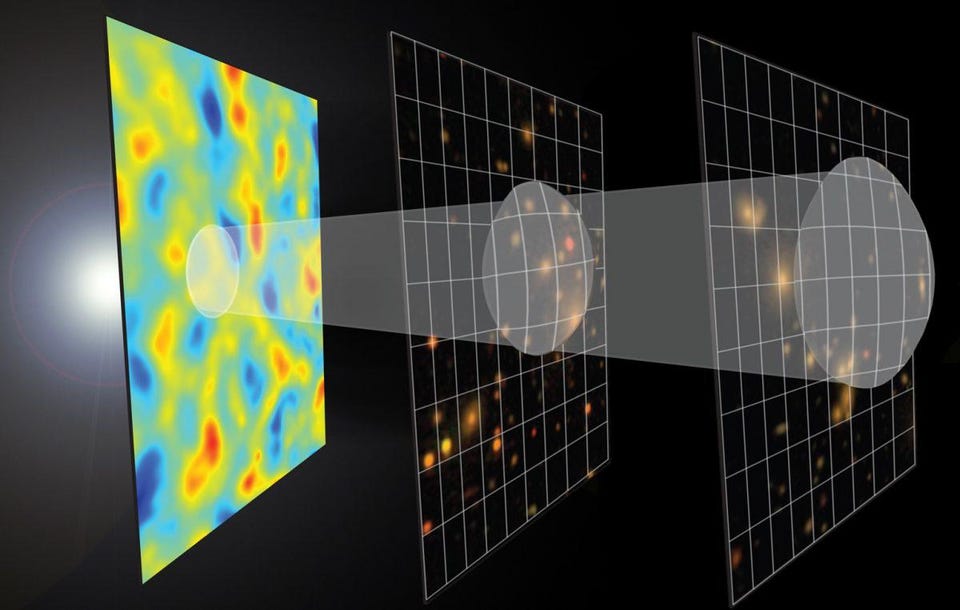
However, the intricate patterns that will eventually manifest within the cosmic web are established much earlier, as the “seeds” of the vast-scale cosmic structure were imprinted during the very early Universe. Stars, galaxies, galaxy clusters, and the filamentary structures spanning the largest scales can all be traced back to density irregularities originating from the time when neutral atoms first materialized in the Universe. These “seeds” would gradually evolve, spanning hundreds of millions and even billions of years, into the intricate cosmic architecture we observe today. These seeds permeate the entire Universe and persist as temperature irregularities in the lingering afterglow of the Big Bang, known as the cosmic microwave background.
As measured by the WMAP satellite during the 2000s and its successor, the Planck satellite, during the 2010s, these temperature fluctuations are observed across all scales, corresponding to density fluctuations in the early Universe. This connection is a consequence of gravity and the principle that, according to General Relativity, the presence and concentration of matter and energy influence the curvature of space. When light traverses from the region of space where it originates to the observer’s vantage point, it results in the following:
- Overdense regions, containing more matter and energy than the average, will appear colder than average, as the light must ascend from a deeper gravitational potential well.
- Underdense regions, with less matter and energy than the average, will appear hotter than average, as the light encounters a shallower gravitational potential well during its journey.
- Regions with an average density will exhibit an average temperature, corresponding to the mean temperature of the cosmic microwave background
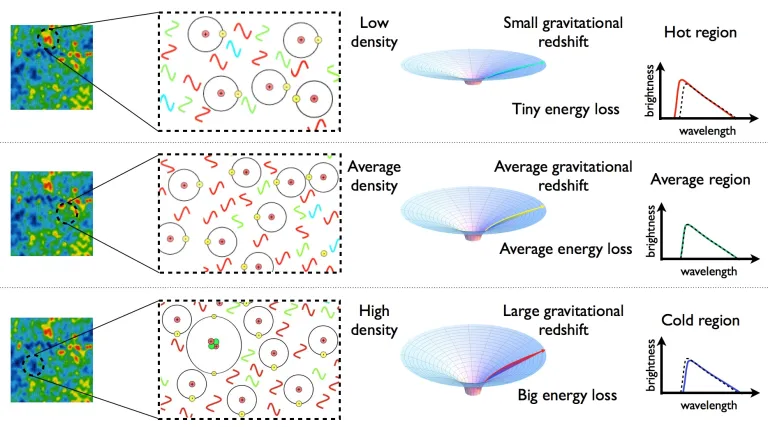
But how did these initial imperfections come into existence? The temperature irregularities we observe in the residual radiation from the Big Bang originated during an epoch that occurred 380,000 years after the onset of the hot Big Bang, which implies that they underwent 380,000 years of cosmic evolution. The explanation varies significantly depending on which perspective you adopt.
According to the “singular” Big Bang hypothesis, the Universe was essentially “born” with an initial set of imperfections. These imperfections subsequently expanded and evolved in accordance with the principles of gravitational collapse, particle interactions, and the interplay of radiation with matter, encompassing the distinctions between normal and dark matter.
On the other hand, according to the inflationary origin theory, where the hot Big Bang emerges only after a period of cosmic inflation, these imperfections originate from quantum fluctuations. These fluctuations arise due to the intrinsic energy-time uncertainty relationship in quantum physics during the inflationary era, characterized by the Universe’s exponential expansion. These quantum fluctuations, originating on the smallest scales, are stretched to larger scales by inflation. Simultaneously, newer fluctuations occurring at later times are superimposed upon them, resulting in a composite of these fluctuations across all distance scales.
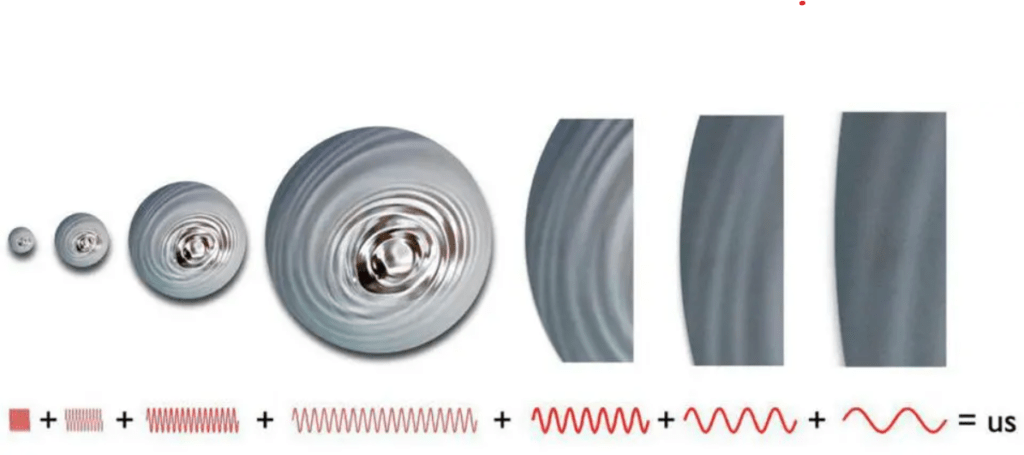
These two conceptual frameworks exhibit fundamental distinctions, but their significance to astrophysicists lies in the potential disparities in the observable signatures they could yield. In the “singular” Big Bang model, the types of fluctuations we would anticipate observing would be constrained by the speed of light. This limitation refers to the maximum distance that any signal, whether gravitational or otherwise, could have traversed if it had propagated at the speed of light within the expanding Universe that originated from a singular event known as the Big Bang.
Conversely, in a Universe that underwent a period of inflation preceding the commencement of the hot Big Bang, we would anticipate density fluctuations across all scales, including those surpassing the distances that a signal could have traveled at the speed of light since the inception of the hot Big Bang. Inflation effectively enlarges the Universe’s size in all three dimensions with each infinitesimal fraction of a second that transpires, leading fluctuations occurring just a few hundred fractions of a second ago to expand to scales larger than the presently observable Universe.
While subsequent fluctuations overlay themselves upon earlier, larger-scale fluctuations, inflation enables us to initiate the Universe with ultra-large-scale fluctuations that would not exist if the Universe commenced from a Big Bang singularity without inflation.
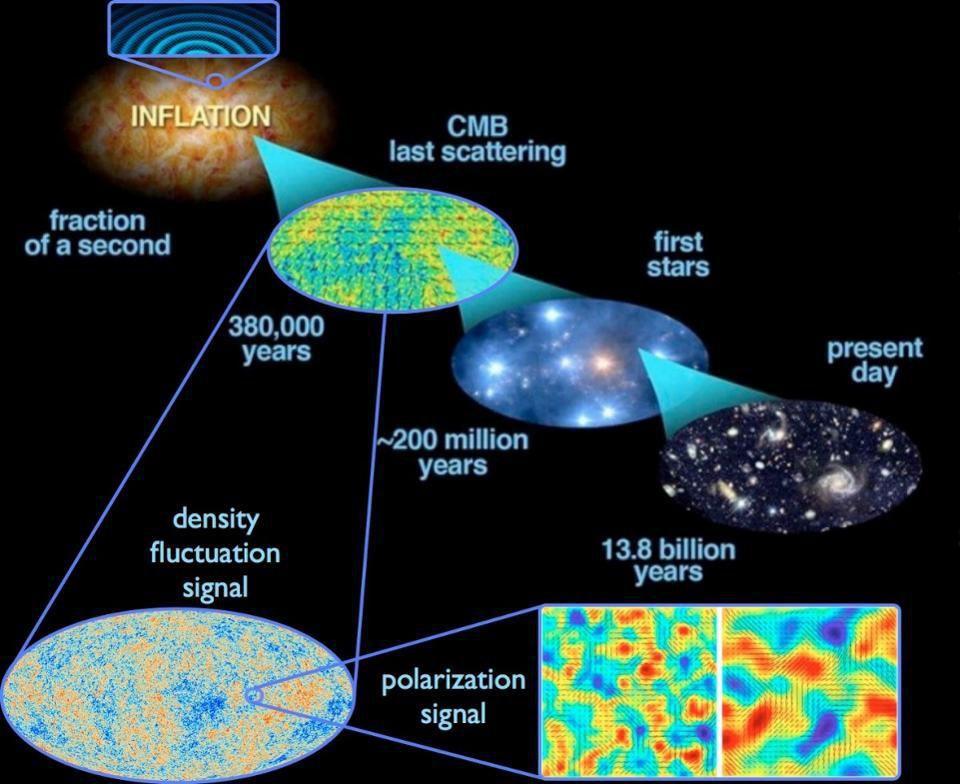
Put simply, a crucial test involves a comprehensive examination of the Universe’s intricate details, specifically to ascertain the presence or absence of a vital element known as “super-horizon fluctuations,” as referred to by cosmologists. Throughout any moment in the Universe’s history, there exists a maximum distance that a signal traveling at the speed of light since the inception of the hot Big Bang could have covered. This distance defines what is known as the cosmic horizon.
- On scales smaller than the horizon, referred to as sub-horizon scales, physical processes can be influenced by events occurring since the onset of the hot Big Bang.
- On scales equivalent to the horizon, labeled as horizon scales, we encounter the upper limit of what could have been impacted by physical signals since the start of the hot Big Bang.
- Scales surpassing the horizon, termed super-horizon scales, extend beyond the range of influence of physical signals generated at or since the commencement of the hot Big Bang.
In essence, the quest to discover signals manifesting on super-horizon scales provides a powerful means to differentiate between a non-inflationary Universe that initiated with a singular hot Big Bang (which should lack such signals entirely) and an inflationary Universe that underwent an inflationary phase preceding the onset of the hot Big Bang (which should exhibit these super-horizon fluctuations).
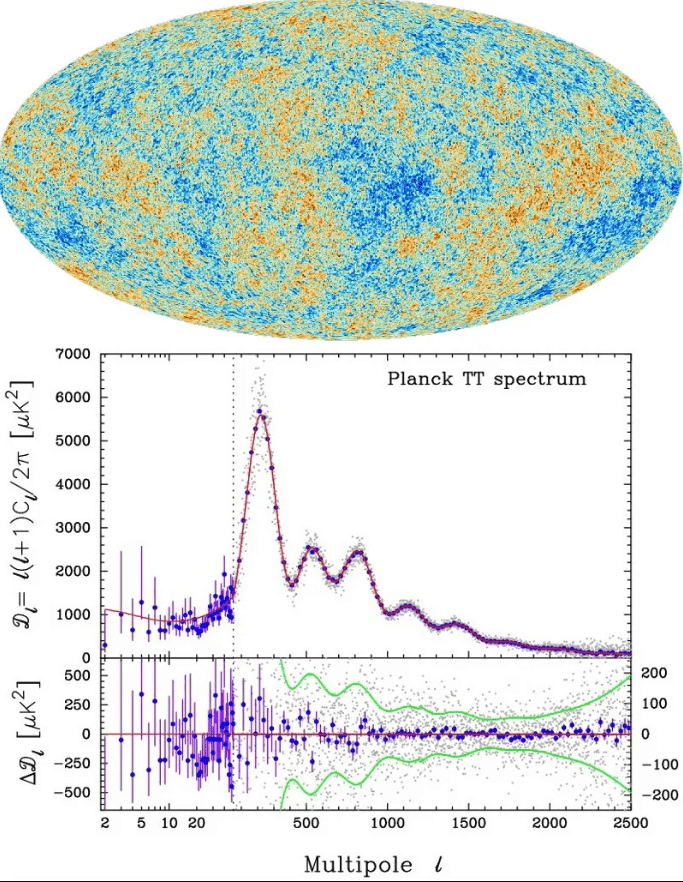
Unfortunately, merely examining a map illustrating temperature fluctuations in the cosmic microwave background doesn’t provide sufficient information to distinguish between these two scenarios. The temperature distribution in the cosmic microwave background can be dissected into various components, some of which span vast angular scales across the celestial sphere, while others span smaller angular scales, and there are intermediary components as well.
The issue lies in the fact that fluctuations on the largest scales could have two potential origins. On one hand, they may have been generated by fluctuations that emerged during an inflationary period. On the other hand, these fluctuations could also be a consequence of the gravitational growth of structures in the Universe at later times, given that the late-time Universe possesses a significantly larger cosmic horizon compared to the early-time Universe.
To illustrate, consider a situation where all that’s available is a gravitational potential well for a photon to ascend from. In this scenario, the photon expends energy during its ascent, a phenomenon known in physics as the Sachs-Wolfe effect. This effect occurs at the point when the photons were initially emitted.
However, if the photon descends into a gravitational potential well during its journey, it gains energy. Subsequently, as it ascends once more on its path towards an observer, it loses energy. If these gravitational imperfections change in size over time, as they do in various ways within a Universe influenced by dark energy and gravitation, different regions of space can exhibit temperatures either hotter or colder than the average, contingent upon the expansion or contraction of density irregularities within that space. This is termed the integrated Sachs-Wolfe effect.
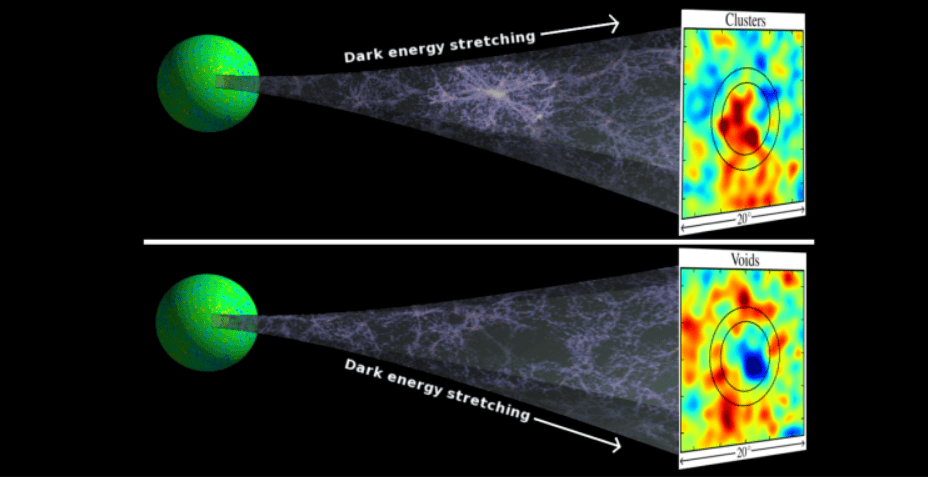
When we examine the temperature irregularities within the cosmic microwave background on these grand cosmic scales, the data alone does not provide sufficient information to determine whether these anomalies were generated by the Sachs-Wolfe effect associated with inflation, if they originated from the integrated Sachs-Wolfe effect due to the growth or contraction of foreground structures, or if they result from a combination of both processes.
Fortunately, we have an additional method to gather insights about the Universe aside from studying the cosmic microwave background’s temperature. We can also analyze the polarization characteristics of the light emitted from the cosmic background. As light traverses the Universe, it interacts with the matter within it, particularly with electrons (remember that light is an electromagnetic wave!). When the light exhibits polarization in a radially symmetric manner, it represents an E-mode (electric) polarization. On the other hand, when the light demonstrates polarization in a clockwise or counterclockwise fashion, it signifies a B-mode (magnetic) polarization.
Nevertheless, solely detecting polarization is insufficient to demonstrate the presence of super-horizon fluctuations.
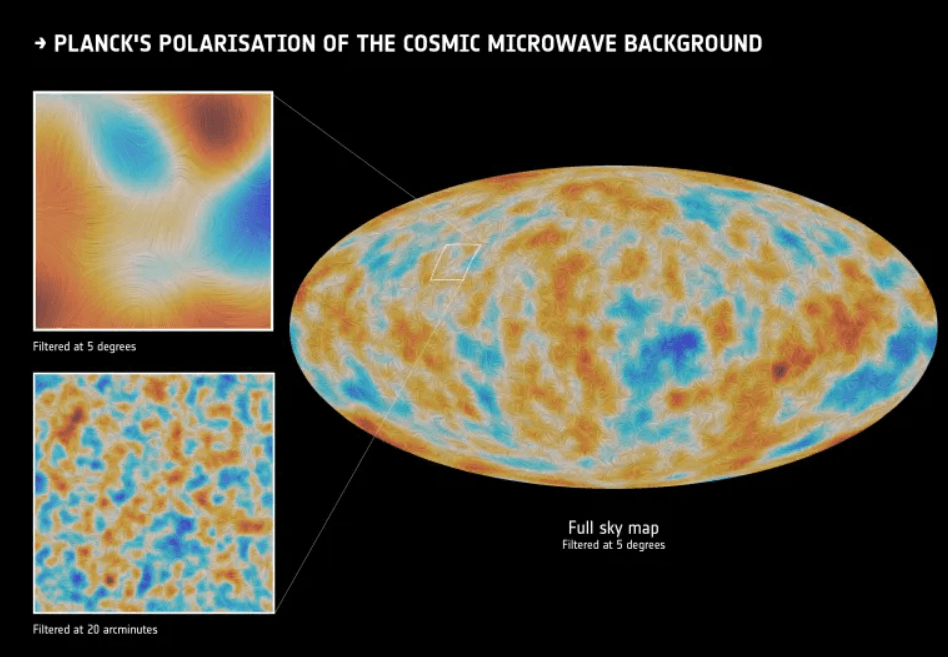
To distinguish between the “singular Big Bang without inflation” and the “inflationary state leading to the hot Big Bang” scenarios, a crucial step is to conduct a correlation analysis. This analysis involves examining the relationship between the polarized light and the temperature fluctuations within the cosmic microwave background and correlating them at the same angular scales. This is the juncture where observational investigations of our Universe become highly intriguing because it enables us to differentiate between these two scenarios.
In both scenarios, we anticipate observing sub-horizon correlations, encompassing both positive and negative correlations, between the E-mode polarization in the cosmic microwave background and the temperature fluctuations within it. Moreover, on the scale of the cosmic horizon, corresponding to angular scales of approximately 1 degree (equating to a multipole moment around l = 200 to 220), we expect these correlations to be non-existent.
However, in the “singular Big Bang” scenario, specific to super-horizon scales, we will observe a solitary, substantial positive correlation between the E-mode polarization and the temperature fluctuations in the cosmic microwave background. This correlation coincides with the period when stars form in substantial quantities and reionize the intergalactic medium. On the other hand, the “inflationary Big Bang” scenario encompasses this positive correlation but also includes a sequence of negative correlations between the E-mode polarization and the temperature fluctuations on super-horizon scales, which span approximately 1 to 5 degrees (corresponding to multipole moments ranging from l = 30 to l = 200).
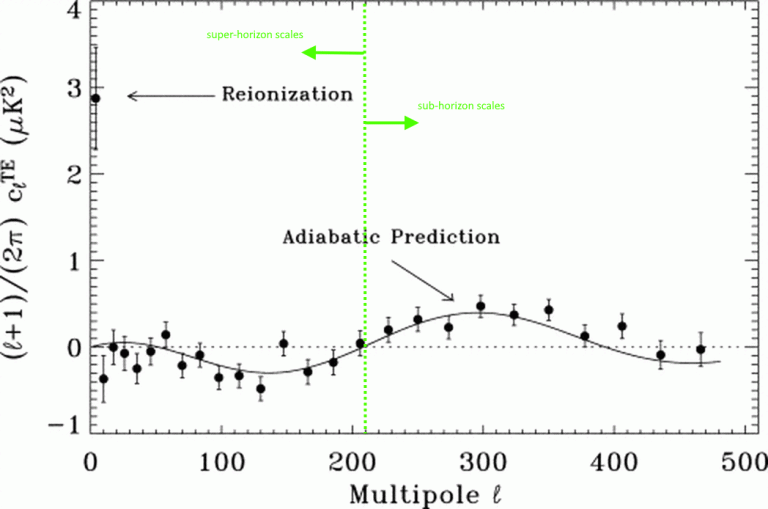
The graph displayed above represents the initial chart, released by the WMAP team back in 2003, a full two decades ago. This chart illustrates what cosmologists refer to as the TE cross-correlation spectrum, which outlines the correlations between the E-mode polarization and the temperature fluctuations found in the cosmic microwave background across all angular scales. I’ve added green annotations to indicate the scale of the cosmic horizon, as well as arrows that signify sub-horizon and super-horizon scales.
Upon closer examination, it’s evident that on sub-horizon scales, both positive and negative correlations are present. However, on super-horizon scales, there is a conspicuous, substantial dip in the data, aligning with the predictions of inflation (represented by the solid line) and unequivocally contradicting the predictions of the non-inflationary, singular Big Bang scenario (represented by the dotted line).
Naturally, that was two decades ago, and the WMAP satellite has since been succeeded by the Planck satellite, which offered numerous enhancements. Planck observed the Universe across a broader range of wavelength bands, examined smaller angular scales, possessed greater temperature sensitivity, included a dedicated polarimetry instrument, and conducted more comprehensive sky surveys, resulting in reduced errors and uncertainties. When we examine the final Planck TE cross-correlation data from the 2018 era, the findings are truly remarkable.
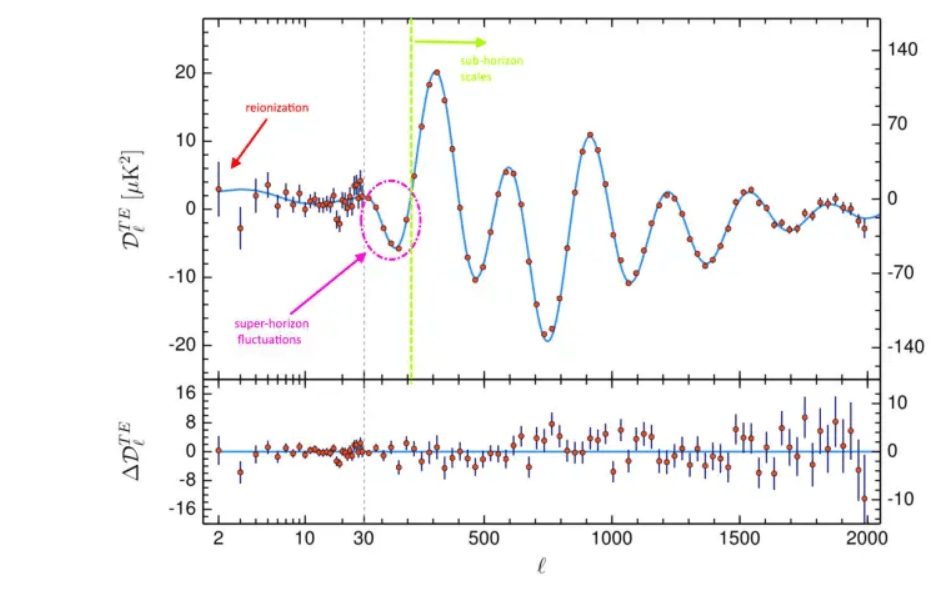
As it becomes evident, there can be no room for doubt regarding the existence of super-horizon fluctuations within the Universe, given the overwhelming significance of this signal. The presence of super-horizon fluctuations, observed not only during reionization but also as anticipated by inflation, provides undeniable evidence: the non-inflationary, singular Big Bang model does not align with the observable Universe. Instead, our observations lead us to conclude that we can trace the Universe’s history back to a specific cutoff point within the framework of the hot Big Bang, and preceding that, an inflationary phase must have been in effect.
While we wish we could provide more insights about the Universe, the observable limits restrict our observations. Fluctuations and imprints on larger scales exert no discernible impact on the Universe we can directly observe. There are additional tests to scrutinize the validity of inflation, such as examining a nearly scale-invariant spectrum of purely adiabatic fluctuations, assessing a maximum temperature cutoff for the hot Big Bang, detecting slight deviations from perfect flatness in the cosmological curvature, and studying the primordial gravitational wave spectrum, among others. However, the super-horizon fluctuation test stands out as a straightforward and exceedingly robust means of confirmation.
On its own, this test unequivocally establishes that the Universe’s origin was not simply the result of a hot Big Bang; rather, it was preceded by an inflationary phase that prepared the groundwork for what we observe today. Though it may not always be recognized as such, this discovery, in and of itself, undoubtedly qualifies as an accomplishment deserving of Nobel recognition.
This article is republished from BigThink under a Creative Commons license. Read the original article.
Do not forget to share your opinion with us to provide you with the best posts !
0 Comments